By combining the principles of microfluidics, microfabrication, and cell culturing techniques, a new generation of laboratory platforms has emerged, known as “organ-on-chip”. Organ-on-chip systems are microfluidic devices containing living cells. These microfluidic devices can better recapitulate microenvironments compared to previous in vitro models. Microfluidic devices are especially suitable for modeling biological barriers, such as the blood-brain barrier, as they can model blood flow.
Structure and function of the blood-brain barrier
The blood-brain barrier (BBB) is the boundary layer of cells that lines the brain micro vessels and separates blood from brain interstitial fluids. The main function of the blood-brain barrier is to act as a physical and metabolic barrier by allowing selective transport of nutrients through, but preventing toxic substances in the blood from entering the brain.
The BBB is made up of specialized endothelial cells which are surrounded by a basement membrane and four other types of cells: astrocytes, pericytes, neurons and microglia. These five types of cells and the basement membrane together make up the neurovascular unit (figure 1) [1]. Sealing protein complexes called tight junctions are formed between adjacent endothelial cells of the BBB. As a result, cells are held in close proximity to each other, thereby occluding the intracellular spaces and preventing paracellular transport. This high barrier tightness is characteristic of the BBB, and crucial for maintaining brain homeostasis. A local disruption of the barrier tightness, resulting in a leakage through the barrier, is associated with several diseases of the central nervous system (CNS) [2]. Understanding and overcoming the BBB is essential for treatment of diseases on the CNS. Drug delivery systems that can temporarily open the barrier, allowing medication to pass through and reach the brain, is a key research area for the treatment of CNS diseases.
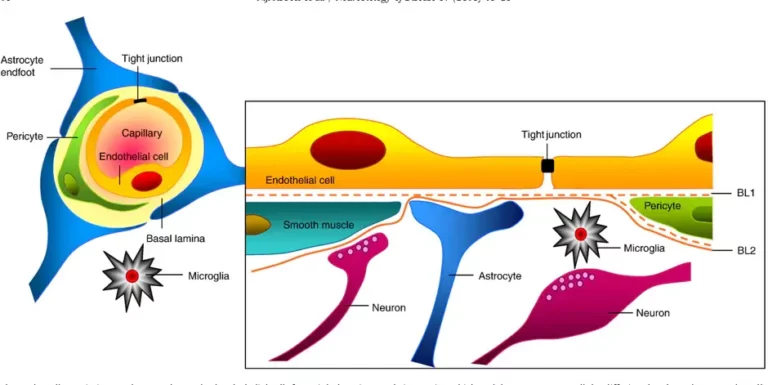
In vitro models of the blood-brain barrier
In artificial environments, conditions can be kept under high control and specific behaviors can be independently studied with little interference from other factors. Traditionally, the BBB has been reconstructed in vitro as static models using conventional cell culture setups. Microfluidic organ-on-chip devices offer solutions to many of the shortcomings of previous in vitro models. These devices can be designed with more realistic architectures and their small dimensions better represent the geometry of the capillaries in the neurovascular unit. Further, by employing microfluidics instead of conducting experiments in a bulk solution, the models can display physiological phenomena such as fluid flows and shear stresses. Another advantage of BBB-on-chip models is that sensors for real-time monitoring can be integrated [3].
Blood-brain barrier on a microfluidic chip
Two of the early microfluidic devices that have pioneered the development of BBB-on-chip and provided proof-of-principle for several promising concepts are presented below. Some important design aspects and validation parameters to consider when developing an organ-on-chip device to study the BBB will also be introduced. Today, ready-to-use chips for organ-on-chip applications are commercially available or can be ordered custom-made.
To our knowledge, Booth et al. were the first to publish a BBB on-chip model in 2012 [4] with a follow-up paper in 2014 [5]. Their device, shown in figure 2, comprises two crossing channels in layers of PDMS, with a depth of 200 µm and a width of 2 mm and 5 mm respectively. The two channels were separated by a porous polycarbonate membrane covered by fibronectin. The channel layers were covered by glass slides with thin film Ag/AgCl electrodes with a four-point sensing structure placed over the membrane. Mouse endothelial cells (b.End3) and murine astrocytes (C8D1A) were cocultured on opposite sides of the membrane. A flow rate of 2.6 mL/min was applied in both channels, which according to calculations presented in the paper, corresponded to a shear stress of 2 mPa on the endothelial cells. The transendothelial electrical resistance (TEER) was measured to 180-280 Vcm2 with the integrated thin film electrodes. Further, the tight junction protein ZO-1 was identified with immunofluorescence, and permeability studies were performed to confirm the barrier function.
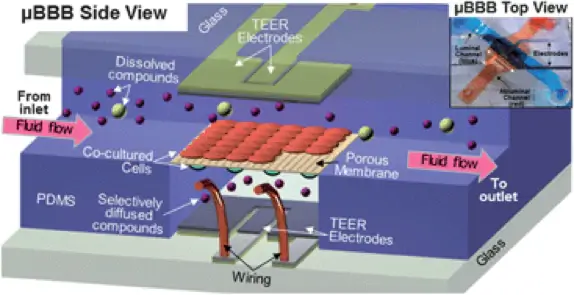
Another fully integrated BBB device was published by Griep et al. in 2013 [6]. The device was made up of two layers of PDMS comprising channels with a width of 500 µm and depth of 100 µm, with a polycarbonate membrane coated with collagen between. Human cerebral microvascular endothelial cells (hCMEC/D3) were cultured on top of the membrane. They used four platinum wires to monitor the TEER value over 7 days from impedance spectroscopy measurements. They obtained an average TEER value of 28 Vcm2 ± 1.3 SEM). They observed an increase in TEER, up to 120 Vcm2, when a flow rate of 2.5 mL/h was applied, corresponding to a calculated shear stress of 0.58 Pa.
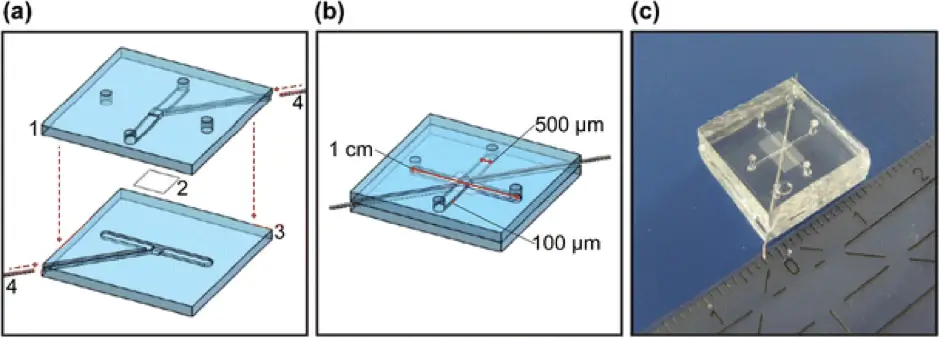
Chip material for organ-on-chip
PDMS has been used extensively to fabricate organ-on-chip devices. Some of the advantages of PDMS include being biocompatible and transparent (240 nm – 1100 nm), cheap and easy to handle, can be molded with high resolution, and can bond to glass or PDMS [7]. It is however highly hydrophobic which can be problematic when filling the channels or obtaining tight bonding for non-leaking chips. Furthermore, it is difficult to deposit electrodes on the surface of PDMS, and glass is therefore often used in devices with patterned thin film electrodes.
Cells in blood-brain barrier on chip models
The type of cells used is of great importance to construct models of biological relevance. It is however still unclear exactly how the different cells of the neurovascular unit contribute to the barrier function [8]. To mimic the human BBB, using human cells would obviously be the most predictive. Human brain capillary endothelial cells have been widely characterized but have low availability and reproducibility [1]. Recent advancements in deriving human induced pluripotent stem cells (hiPSC) hold great promise to overcome such difficulties [9].
Co-culturing endothelial cells with other cells from the neurovascular unit are expected to be a more physiologically relevant representation, as these cells also influence the formation and maintenance of the barrier [10]. Several studies of co-cultures in microfluidic BBB models have already been published, mainly endothelial cells with astrocytes [4] [11], but also with neurons [12] and pericytes. Astrocytes and endothelial cells are not in direct contact with each other but are separated by the basal lamina. In microfluidic devices, they can be cultured on separate sides of the membrane. However, if the membrane in the device is too thick, the cell-cell contact will be limited. To mimic the 3D environment of the extracellular matrix, hydrogels have been used as cell-seeding scaffolds inside microfluidic chambers [12].
Shear stress on endothelial cells
The endothelial cells of the BBB in the neurovascular unit are subjected to a force normal to the vessel wall due to blood pressure, as well as shear stress resulting from the blood flow, in the direction of the flow. Shear stresses have already been reported to influence endothelial cell morphology and function, and to have a positive effect on barrier formation [13]. Organ-on-chip devices have the great advantage of being suited for the incorporation of fluid flow, unlike other types of devices. Living blood vessels have circular cross-sections, while microfluidic devices typically have rectangular cross-sections. In a tube with a circular cross-section, the shear stress will be equal and uniform on the walls due to the cylindrical symmetry. In the case of a rectangular cross-section, however, the shear stress will not be uniform. To achieve the most uniform stress in a rectangular cross-section, a flow profile as flat as possible can be achieved by designing the channels to have a channel height much smaller than the channel width [14]. The physiological shear stress for brain capillaries is 0.3-2 Pa [2].
Transendothelial electrical resistance (TEER)
TEER describes the resistance across a cellular barrier and is often used as a validation parameter to control if endothelial cells in BBB models form tight junction complexes [15]. If the endothelial cells are growing properly and form tight junctions to adjacent cells, the intercellular spaces of the cell layer are occluded and the flow of current through is restricted [16]. This induces a higher resistance to current, which is reflected in higher TEER values. TEER is often expressed normalized to the membrane area in the unit Ωcm2, as in the below, to facilitate direct comparison to other models.
𝑇𝐸𝐸𝑅 (Ω 𝑐𝑚2) = 𝑅𝑒𝑠𝑖𝑠𝑡𝑎𝑛𝑐𝑒 (Ω) × 𝐶𝑒𝑙𝑙 𝑐𝑢𝑙𝑡𝑢𝑟𝑒 𝑎𝑟𝑒𝑎 (𝑐𝑚2)
In BBB on-chip models, measuring TEER is a quick, non-invasive method enabling real-time evaluation of the quality of the barrier. The measurements are performed by applying a low electrical current across the cellular layer while measuring how much the current is impeded by the cell layer. Organ-on-chip devices are suitable for integrating sensors where TEER can be monitored in real-time, which is difficult in other types of devices. In vitro models of the BBB need to reach TEER values above 150-200 Ωcm2 to be considered acceptable models [19]. This is approximately a factor ten lower than the reported in vivo results. The reason why a much lower TEER is accepted is due to the simplified conditions in vitro.
Permeability of blood-brain barrier on chip models
Organ-on-chip models can directly provide information about the barrier function by testing the permeability of the barrier to different substances. It is useful to quantify the permeability in a way that it can be easily compared to in vivo data. Passive transport across the barrier can be quantified from the permeability coefficient of an analyte flowing through the chip. It should be noted that factors other than diffusion may contribute to the transport, such as convective flow if there are gaps in the cell barrier, and osmotic flow due to differences in solute concentrations between the channels. This can be avoided by controlling the solute concertation in the channels and having the same pressure in both channels during the experiments. As the transport of hydrophilic compounds across the barrier is very restricted, these compounds labeled with fluorescent tracers are often used for permeability studies. The permeability can then be visualized with a fluorescent microscope.
Written by Emma Thomée, PhD student for the MAMI Project, with funding from the European Union’s Horizon 2020 research and innovation programme under grant agreement No. 766007.
Bibliography
- Abbott, N.J., et al., Structure and function of the blood-brain barrier. Neurobiol Dis, 2010. 37(1): p. 13-25.
- Wong, A.D., et al., The blood-brain barrier: an engineering perspective. Front Neuroeng, 2013. 6: p. 7.
- Bhatia, S.N. and D.E. Ingber, Microfluidic organs-on-chips. Nat Biotechnol, 2014. 32(8): p. 760-72.
- Booth, R. and H. Kim, Characterization of a microfluidic in vitro model of the blood-brain barrier ([small mu ]BBB). Lab on a Chip, 2012. 12(10): p. 1784-1792.
- Booth, R. and H. Kim, Permeability analysis of neuroactive drugs through a dynamic microfluidic in vitro blood-brain barrier model. Ann Biomed Eng, 2014. 42(12): p. 2379-91.
- Griep, L.M., et al., BBB on chip: microfluidic platform to mechanically and biochemically modulate blood-brain barrier function. Biomed Microdevices, 2013. 15(1): p. 145-50.
- Sia, S.K. and G.M. Whitesides, Microfluidic devices fabricated in poly(dimethylsiloxane) for biological studies. Electrophoresis, 2003. 24(21): p. 3563-76.
- Hawkins, B.T. and T.P. Davis, The Blood-Brain Barrier/Neurovascular Unit in Health and Disease. Pharmacological Reviews, 2005. 57(2): p. 173.
- Pamies, D., T. Hartung, and H.T. Hogberg, Biological and medical applications of a brain-on-a-chip. Experimental Biology and Medicine, 2014. 239(9): p. 1096-1107.
- Serlin, Y., et al., Anatomy and Physiology of the Blood-Brain Barrier. Seminars in cell & developmental biology, 2015. 38: p. 2-6.
- Bang, S., et al., A Low Permeability Microfluidic Blood-Brain Barrier Platform with Direct Contact between Perfusable Vascular Network and Astrocytes. Scientific Reports, 2017. 7(1): p. 8083.
- Adriani, G., et al., A 3D neurovascular microfluidic model consisting of neurons, astrocytes and cerebral endothelial cells as a blood-brain barrier. Lab On A Chip, 2017. 17(3): p. 448-459.
- Dewey, C.F., et al., The dynamic response of vascular endothelial cells to fluid shear stress. J Biomech Eng, 1981. 103(3): p. 177-85.
- van der Helm, M.W., et al., Microfluidic organ-on-chip technology for blood-brain barrier research. Tissue Barriers, 2016. 4(1): p. e1142493.
- Srinivasan, B., et al., TEER measurement techniques for in vitro barrier model systems. J Lab Autom, 2015. 20(2): p. 107-26.
- Thuenauer, R., E. Rodriguez-Boulan, and W. Römer, Microfluidic approaches for epithelial cell layer culture and characterisation. Analyst, 2014. 139(13): p. 3206-18.
- Benson, K., S. Cramer, and H.J. Galla, Impedance-based cell monitoring: barrier properties and beyond. Fluids Barriers CNS, 2013. 10(1): p. 5.
- Wegener, J. and J. Seebach, Experimental tools to monitor the dynamics of endothelial barrier function: a survey of in vitro approaches. Cell Tissue Res, 2014. 355(3): p. 485-514.
- Reichel, A., D.J. Begley, and N.J. Abbott, An overview of in vitro techniques for blood-brain barrier studies. Methods Mol Med, 2003. 89: p. 307-24.